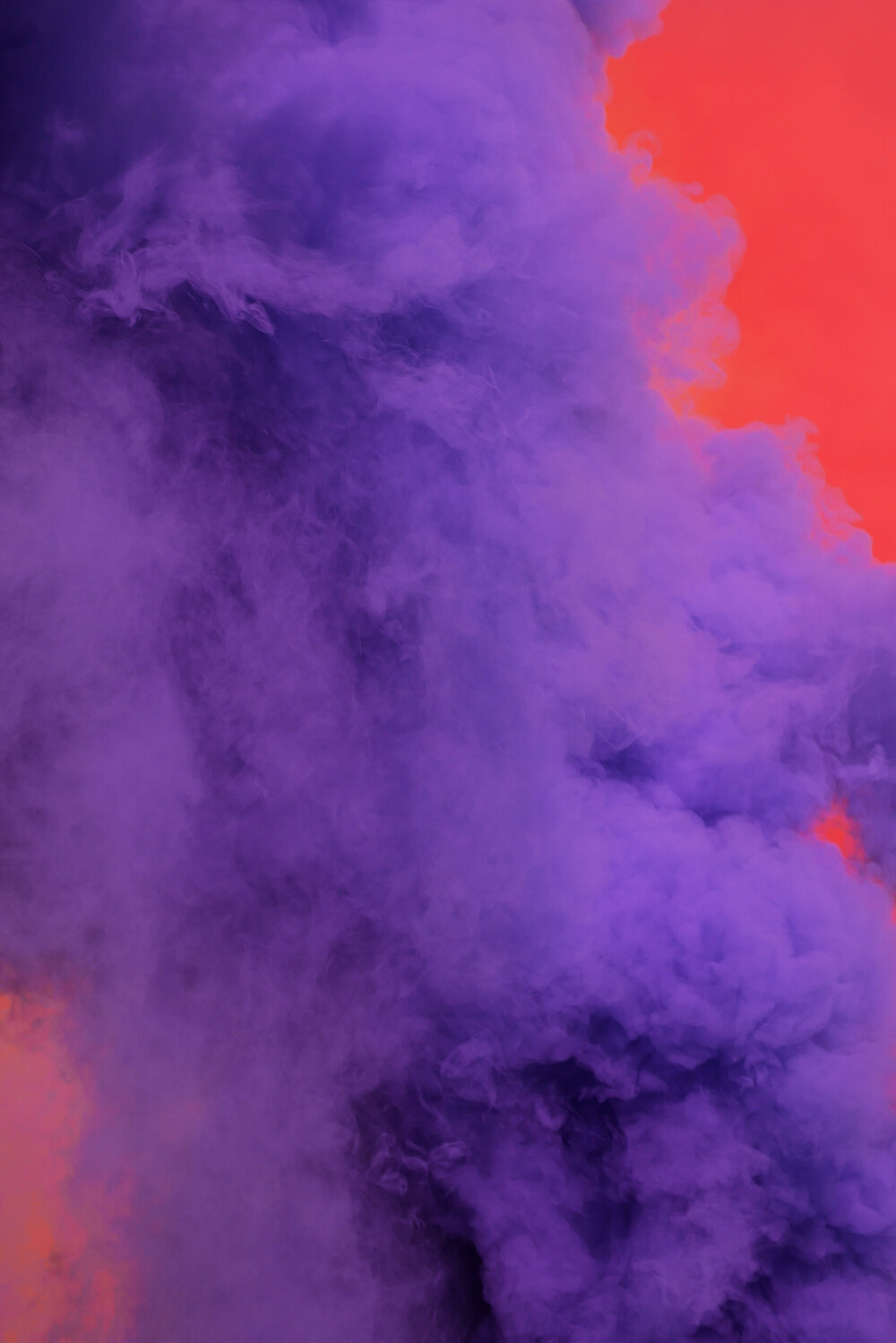
See below two descriptions of my research activities—one aimed towards physicists, and the other aimed towards the general public..
For Physicists
Me in the McKinsey lab in Birge Hall at UC Berkeley. This is our “helium room”, in which we build experiments to understand the nature of energy depositions in liquid helium.
I am a member of the dark matter direct detection community. Specifically, I use liquid noble elements (helium and xenon) as targets to search for dark matter-induced nuclear recoils. I am a member of Professor Dan McKinsey’s group at the University of California, Berkeley and affiliated with the dark matter group at Lawrence Berkeley National Laboratory. From 2018-2020, I was the inaugural recipient of the Graduate Instrumentation Research Award, funded by the U.S. Department of Energy’s Office of High Energy Physics and organized by Fermilab and the Coordinating Panel for Advanced Detectors.
I am a member of the SPICE/HeRALD collaboration, a group working to detect sub-GeV/c^2 dark matter via cryogenic targets and transition-edge sensor (TES) readout. A description of the project is here. Within this collaboration, I am closely affiliated with the Helium Roton Apparatus for Light Dark Matter (HeRALD) project, focusing on a superfluid helium target. Our idea paper is here, which describes the benefits of using helium. One key benefit is the use of calorimetry for signal readout, since helium stays a liquid/superfluid down to absolute zero temperature. Quanta of heat, observed as phonons and rotons, can be observed via quantum evaporation of helium atoms into vacuum and subsequent adsorption of the atoms onto a calorimter wafer. This technique should allow us to probe dark matter with masses of 𝒪(MeV/c^2) or even lower. In the paper linked above, one of my primary responsibilities was calculating the sensitivity of the detector to dark matter interactions and extending the neutrino floor to lower dark masses. Currently our group at Berkeley is working to better understand how energy depositions in liquid helium manifest themselves, i.e. their light and heat yields. At left (or below, on mobile), you can see a picture of our lab at UC Berkeley! For the past several months, I have been working with Aritoki Suzuki at LBNL to run a dilution fridge, in which we are testing the performance of TES sensors.
Within the world of xenon detectors, I am a member of the Large Underground Xenon (LUX), Noble Element Simulation Technique (NEST), and Particle Identification in Xenon at Yale (PIXeY) collaborations. LUX was a two-phase liquid/gas xenon time projection chamber which ran from 2013-16; I have been a member since 2016 and an author since 2017. The collaboration set then-world-leading limits on WIMP-nucleon spin-independent interactions, as well as limits on other physics processes, such as spin-dependent scatterings, axion-like particles, and nuclear recoils that mimic electronic interactions through the Migdal effect. My main effort on LUX has been in studying the discrimination of electronic vs. nuclear recoils in liquid xenon. I led a comprehensive analysis to study charge-to-light-ratio and pulse-shape discrimination in xenon, and how they are affected by detector parameters like the drift electric field and light collection efficiency. This analysis showed a background rejection of > 10⁵ at the highest energies we considered, two orders of magnitude better than the discrimination power typically associated with this technology in the low-energy regime. The paper is available here.
A generic picture of energy depositions in noble element media; the character A represents any noble element. For a given energy deposit, energy can be detected through heat, charge, and light; the ratio of these and the efficiency of measuring them is critical to any particle physics experiment. The majority of my research is devoted to understanding and detecting the strength of these different channels in xenon and helium.
NEST is a collaboration of noble element physicists (mostly xenon, but some members from the argon community as well) who develop code to simulate energy depositions in detectors. This includes semi-empirical models for signal yields, electron-ion recombination, and detector effects. I have been a member since 2017 and have contributed to development of the electronic recoil model—betas and gammas—and the {83m}Kr model. Our code is available for download on Github, and changes have been tracked on Zenodo.
Finally, I also work on analysis for PIXeY. This is a xenon time projection chamber originally installed at Yale. The experiment is not currently operational, but using older data, we are able to study the behavior of liquid xenon detectors under a variety of operating conditions. I am conducting an ongoing analysis of ER/NR discrimination, complementary to the LUX analysis described above.
For Non-Physicists
A simulation of a galaxy rotating, in the presence of dark matter (left) and without any dark matter (right). The galaxy rotates faster if there is extra hidden dark matter. Astronomers have observed that galaxies behave like the left graphic, providing one piece of evidence for dark matter.
The most exhilarating puzzle in modern physics (in my opinion) is that for all our successes, we still don’t know what most of the universe is made of. 85% of the matter in the universe is “dark”, meaning that it doesn’t interact with light. We know that it’s there, though, because it interacts with gravity—we can see its effects on the speed of galaxies’ rotation, the deflection of light by galaxies and galactic clusters, and even the evolution of the universe as a whole.
As a particle physicist, I’m interested in the nature of dark matter at the most fundamental level. What is dark matter made of? It’s not composed of atoms or their constituents (protons, neutrons, and electrons). It’s not composed of some of the more exotic particles we’ve observed, such as neutrinos. But it is almost certainly made of some individual particle or particles. (If you don’t believe me, XKCD has a succinct if snarky response.)
So what is it? No one knows, but there are ideas! One of the most popular is called the Weakly Interacting Massive Particle, or WIMP. In this hypothesis, dark matter is made of fundamental particles around the mass of 100 protons—the same mass as a silver atom. These particles can interact very rarely with normal atoms through the Weak Nuclear Force. If WIMPs are real, there’s a bunch of them! In every square meter of space on Earth, there should be about 300 WIMPs. But as mentioned, they only rarely collide with matter. Over the course of your lifetime, you might only have one or two atoms in your body be hit by a WIMP; the rest will just pass through as if you weren’t even there.
The central chamber of the upcoming LUX-ZEPLIN (LZ) experiment. This will be filled with liquid xenon, shielded inside a 228-ton water tank, and installed 1 mile underground in an old South Dakota gold mine. LZ will be able to test a larger range of dark matter models than any previous experiment. (I am not a member of the LZ collaboration, but most members of my research group are.)
To figure out what dark matter is, we build some of the most sensitive experiments in the world. The general principle is this: we assemble a large mass of some material (for example, liquid xenon), instrument it with particle detectors that can detect collisions in the material, and monitor it for a length of time (depending on the particular study, this could be days, weeks, or years). If dark matter can scatter off atomic nuclei, and if dark matter particles are passing through our solar system, then this experiment should be able to observe these interactions. This is obviously a very simple picture—complications include noise from other background interactions, the purity of the material, and the efficiency of the detectors. So we also ensure that the experiment is incredibly clean, pure of both chemical and radioactive contaminants, and shielded from external radiation. This latter criteria can be met quite robustly by going deep underground; the Earth’s crust shields our experiment from cosmic radiation. A lot of dark matter experiments are located a mile or more underground in mines!
My research specifically focuses on the use of liquid xenon and liquid helium to search for dark matter. I focus on understanding the detectors and instrumentation we use to build these experiments. This includes studying the internal physics of noble elements and different technologies for particle detection. We have yet to observe dark matter interactions, but even the lack of a discovery is informative by allowing us to reject various physics models. The search continues!